النبات
مواضيع عامة في علم النبات
الجذور - السيقان - الأوراق
النباتات الوعائية واللاوعائية
البذور (مغطاة البذور - عاريات البذور)
الطحالب
النباتات الطبية
الحيوان
مواضيع عامة في علم الحيوان
علم التشريح
التنوع الإحيائي
البايلوجيا الخلوية
الأحياء المجهرية
البكتيريا
الفطريات
الطفيليات
الفايروسات
علم الأمراض
الاورام
الامراض الوراثية
الامراض المناعية
الامراض المدارية
اضطرابات الدورة الدموية
مواضيع عامة في علم الامراض
الحشرات
التقانة الإحيائية
مواضيع عامة في التقانة الإحيائية
التقنية الحيوية المكروبية
التقنية الحيوية والميكروبات
الفعاليات الحيوية
وراثة الاحياء المجهرية
تصنيف الاحياء المجهرية
الاحياء المجهرية في الطبيعة
أيض الاجهاد
التقنية الحيوية والبيئة
التقنية الحيوية والطب
التقنية الحيوية والزراعة
التقنية الحيوية والصناعة
التقنية الحيوية والطاقة
البحار والطحالب الصغيرة
عزل البروتين
هندسة الجينات
التقنية الحياتية النانوية
مفاهيم التقنية الحيوية النانوية
التراكيب النانوية والمجاهر المستخدمة في رؤيتها
تصنيع وتخليق المواد النانوية
تطبيقات التقنية النانوية والحيوية النانوية
الرقائق والمتحسسات الحيوية
المصفوفات المجهرية وحاسوب الدنا
اللقاحات
البيئة والتلوث
علم الأجنة
اعضاء التكاثر وتشكل الاعراس
الاخصاب
التشطر
العصيبة وتشكل الجسيدات
تشكل اللواحق الجنينية
تكون المعيدة وظهور الطبقات الجنينية
مقدمة لعلم الاجنة
الأحياء الجزيئي
مواضيع عامة في الاحياء الجزيئي
علم وظائف الأعضاء
الغدد
مواضيع عامة في الغدد
الغدد الصم و هرموناتها
الجسم تحت السريري
الغدة النخامية
الغدة الكظرية
الغدة التناسلية
الغدة الدرقية والجار الدرقية
الغدة البنكرياسية
الغدة الصنوبرية
مواضيع عامة في علم وظائف الاعضاء
الخلية الحيوانية
الجهاز العصبي
أعضاء الحس
الجهاز العضلي
السوائل الجسمية
الجهاز الدوري والليمف
الجهاز التنفسي
الجهاز الهضمي
الجهاز البولي
المضادات الحيوية
مواضيع عامة في المضادات الحيوية
مضادات البكتيريا
مضادات الفطريات
مضادات الطفيليات
مضادات الفايروسات
علم الخلية
الوراثة
الأحياء العامة
المناعة
التحليلات المرضية
الكيمياء الحيوية
مواضيع متنوعة أخرى
الانزيمات
Cell Lineage
المؤلف:
J. B. L. Bard
المصدر:
Morphogenesis: The Cellular and molecular processes of Developmental Anatomy
الجزء والصفحة:
20-12-2015
4268
Cell Lineage
The daunting complexity of vertebrate organisms has led investigators to adopt a reductionist approach to their investigation. This approach is based on the assumption that an otherwise impossibly complicated system can be effectively understood through an analysis of its constituent cells and molecules. A reductionist concept of development envisions the process as the assembly of elementary units in many combinations and permutations, unfolding over time in stages that escalate progressively in their complexity. The transition from one stage to the next is influenced by the conditions that prevailed during the earlier period. Developmental biology thus seeks to infer the mechanisms of morphogenesis and histogenesis from the properties, activities, and responses of the precursors that give rise to the various lineages of the cells of the body.
That the concept of lineage should be an important one in developmental biology is something of a paradox. In a simplistic sense, it seems odd that lineage can be a meaningful issue, when all of the cells of an animal descend from a single precursor, the fertilized zygote. Distinct lineages, however, arise very early in embryogenesis. Formation of a body plan in lower vertebrates, including amphibia, is a process that begins in the oocyte, continues after fertilization and cleavage, and is finally completed at gastrulation (1, 2). Polarity in the oocyte causes cytoplasmic constituents to be distributed asymmetrically in the egg and newly formed blastula (3-6). This asymmetric distribution establishes different lineages in blastomeres, which do not each inherit identical cytoplasmic determinants from their predecessors. The resulting differential inheritance creates cellular differences between the blastomere-derived lineages and allows cells to interact with one another (7, 8). Regional inequalities and cellular interactions may then be expressed as functional differences between cells in different locations. These processes form an ordered cascade that leads to the establishment of a pattern of small groups of like cells that follow a developmental agenda common to themselves, but different from those followed by other small groups of cells located elsewhere in the embryo (4). Functional differences between cells located in particular sites in the developing embryo are necessary for the differential cellular behaviors that underlie morphogenetic movements, like gastrulation and neurulation (9-11). It is also these different cellular loci, and the progeny to which they give rise, that provide developmental meaning to the concept of lineage.
In mammals, the establishment of lineages is delayed relative to that in amphibian embryos. Early cleavage cells of mammalian embryos appear to be identical to one another and totipotent (12). Each of the blastomeres of a two-cell embryo is capable of generating a complete adult, and will do so if the embryo is split (13, 14). Destruction of a single blastomere, moreover, may not prevent the normal development of a mature animal. Chimeric animals can also be constructed by combining morulae (15). It is thus unlikely that localized determinants play a role in mammalian development prior to or during the eight-cell stage. The pattern of cell diversification must be generated at subsequent stages, through the interactions of cells with one another and with their microenvironment.
1. Lineage as a Pedigree
Lineage, understood as a set of cells for which the ancestry can be traced back to a single progenitor, can possibly be delineated in simple transparent embryos, such as that of Caenorhabditis elegans by direct observation (16, 17). Cells can be recognized and followed from egg to adult in a living embryo as they divide, migrate, differentiate, and/or die. Keeping track of the cells is facilitated by the fact that nematode development is virtually invariant. Because the process is so constant, it is possible to predict the fates of descendant cells from their positions on the lineage tree. It is, of course, not possible to use similar direct observation by itself to determine the pedigrees of the cells of an adult mammal. Not only are the numbers of cells in a mammalian embryo too large to permit the progeny of unmarked individual cells to be followed, the details of cell lineage in mammals may also show random variations, even between genetically identical individuals. Lineage can possibly be followed in mammals by marking individual precursor cells in one or another location, for example, with a replication-deficient retrovirus that carries the gene encoding Escherichia coli beta-galactosidase (18-20). The activity of the bacterial enzyme or the protein can then be detected histochemically or immunocytochemically, respectively, thereby permitting all of the cells of the clone that ultimately develops from the single virus-infected progenitor to be identified. This type of analysis has been especially powerful in studies of the development of the central nervous system (CNS).
Studies of C. elegans have been particularly important in establishing that the terminally differentiated cells of an adult animal do not necessarily constitute the lineal descendants of a single founder cell (16, 17, 21). Different phenotypic classes of cells (with the exception of intestinal and germ cells) in C. elegans thus arise from several founder cells, which themselves are found on different arms of the lineage tree. The descendants of a single primitive embryonic precursor, therefore, may become cells of the hypodermis, musculature, and nervous system of the mature animal (21). Lineage in the straightforward pedigree sense thus does not predict the commitment of multipotent precursor cells to a specific phenotype. That choice, the moment when a cell's fate has become determined, may be made long before the terminally differentiated phenotype becomes evident in the appearance of the cell, and may be shared by cells originating from more than a single progenitor (22). Determination in this sense implies that a cell has undergone a lasting and autoperpetuating change that will forevermore distinguish that cell and its progeny from other kinds of cell and will commit the determined cell and its descendants to a common specialized developmental program. Cells may become determined either before, or when, they overtly differentiate (display their ultimate phenotype in a detectable manner); moreover, all of the cells that
are determined to follow a shared developmental program do not necessarily arise from a common precursor, and multipotent precursor cells may divide asymmetrically so that not all of their progeny are identically determined. The observation that cells of a particular terminally differentiated phenotype may not share an origin from a single “founder” has led the concept of lineage to acquire a meaning distinct from its linkage to cellular pedigrees.
2. Lineage and Determination
Cells of embryos that share a determined fate, as well as those that descend from a common founder, are often referred to as members of a common lineage. For example, the multipotent stem cells that are found in the neuroepithelium of the neural tube are self-sustaining, but they also give rise to progeny determined to be neurons or glia; following determination, the cells are said to be developing in neuronal or glial lineages (23). Lineage is used to denote their common determined phenotype, not their origin from a common precursor. The neuroepithelial stem cells proliferate in response to the actions of defined mitogens, including epidermal growth factor (EGF) and fibroblast growth factor (FGF), and differentiate as neurons or glia under the influence of a variety of microenvironmental factors. Factors that have been found under at least some circumstances (in vitro or in vivo) to promote the restriction of subsets of central nervous system stem cells to the glia lineage include sonic hedgehog (Shh) (24), ciliary neurotrophic factor (CNTF) (25), leukemia inhibitory factor (LIF) (25), and bone morphogenetic protein 4 (BMP4) (26).
The generation of the phenotypic diversity of cells in various brain regions occurs in stages, progressing from pluripotentiality to lineage restriction (a phenomenon that prevents cells from giving rise to certain types of progeny), to the determination of specific phenotypes, and finally to the manifestation of the features of the terminally differentiated cells (27). Because of their responsiveness to their microenvironment, transplanted stem cells can integrate into a variety of brain regions, and thus potentially may provide a tool for treating the diseased or injured brain (23). The progenitor cells of the vertebrate brain, however, are themselves heterogeneous and thus members of different lineages (27). These differences help to explain how and when neuroepithelial cells begin to respond to the molecular signals provided by their microenvironment. Environmental factors, be they mitogens, trophic growth factors, or morphogens, can only affect cells that have prepared themselves to be affected. To respond to an extracellular factor, the responding cell must have previously acquired the relevant receptors. Most of the stem cells of the vertebrate nervous system express the intermediate filament protein, nestin (23, 25). Lineages of progenitors, however, can be defined by the expression in common of molecular properties that distinguish the cells of the lineage from other progenitors in the neuroepithelium; such lineage-defining molecular traits include expression of the achaete/scute family of basic helix–loop–helix (bHLH) transcription factors, EGF receptors, ventricular zone gene 1 (vzg-1), and the embryonic neural cell adhesion molecule (E-NCAM) (27). Progenitors in different locations may respond differently to the gliogenesis-promoting factors noted above.
3. Lineages in the Development of the Neural Crest
A source of precursor cells in developing vertebrates that has been extremely valuable for studies of the role(s) of cell lineage in development has been the neural crest (28, 29). This is a transitory structure that appears during embryogenesis and disappears as its component cells disperse, migrating through the embryo to give rise to a wide variety of terminally differentiated cell types (30, 31) . The lineages of cells as diverse as melanocytes, fibroblasts, endocrine cells, smooth and skeletal muscle, cartilage, bone, meningeal cells, Schwann cells, satellite cells, enteric glia, autonomic, enteric, and sensory neurons can all be traced back to the neural crest. A great deal of investigation has been devoted to understanding when crest-derived cells become determined, and what factors cause them to become so. Considerable evidence indicates that at least some of the cells of the premigratory crest are pluripotent. Clones of these cells give rise in culture to several different classes of terminally differentiated cells (32-35). Lineage studies that have marked (by microinjection) individual cells of the premigratory crest (36, 37), as well as early-migrating crest-derived cells (38), have shown that progeny of the marked precursors reach different destinations and thus give rise to a variety of cell types. Furthermore, cultures of stem cells can be prepared from the neural crest (39, 40), just as they can from the neuroepithelium of the neural tube (23, 25).
Although it is clear that the neural crest contains multipotent cells, the population of cells in the premigratory crest is actually heterogeneous. Some of the cells, even in the premigratory crest itself, are already determined (41). Cells determined to develop in the melanocytic lineage, for example, migrate selectively along the dorsolateral pathway (42). Among the uncommitted cells, there appears to be a progressive loss of developmental potential that occurs as a function of age (28, 29, 43). Whether the loss of developmental potential is simply determined by the age of the cells, or by the signals the cells receive as a result of their position in the embryo, has yet to be determined.
Some of the crest-derived cells that arrive in the organs that lie at the ends of their migratory pathways are still multipotential when the crest-derived cells arrive. The bowel has been carefully investigated in this regard and is a good model to illustrate the role of lineages in neural crest development (44). Two techniques have been employed to investigate the developmental potential of the crest-derived cells that colonize the gut. One, back-transplantation, consists of placing a segment of avian gut that has received a complement of émigrés from the neural crest in a migration pathway of a younger host embryo (45, 46). When this is done, crest-derived cells leave the grafts and remigrate in their new hosts. Where they go depends on where the grafts are placed. If the grafts are situated so as to replace the host's crest in locations that normally provide crest-derived émigrés for the bowel, the donor cells migrate to the host's gut and participate in forming the enteric nervous system (ENS). On the other hand, if the grafts are placed anywhere else in the embryo, the enteric crest-derived cells of the donor fail to migrate to the host's bowel. Instead, they migrate to peripheral nerves, sensory, and sympathetic ganglia. Donor cells that arrive in sympathetic ganglia become catecholaminergic sympathetic neurons, even though catecholaminergic neurons are not found in the enteric ganglia of the avian gut. Donor cells that find themselves in peripheral nerves develop as Schwann cells, even though the ENS contains glia rather than Schwann cells. Enteric crest-derived cells thus learn nothing from their previous journey to the bowel and evidently remember nothing of it. Crest-derived cells from the donor gut, however, never give rise to muscle, melanocytes, or connective tissue, indicating that they have lost at least some of developmental potential of their ancestors; nevertheless, they remain pluripotent to the extent that they are able to give rise to cell types that they would not have done had they been left in situ.
Clonal analysis of crest-derived cells isolated from the embryonic avian (47) or fetal mammalian bowel (48, 49) has also suggested that the population of crest-derived émigrés that colonizes the gut is a heterogeneous mixture of multipotent and determined cells. One or more terminally differentiated types of cells may be found in clones arising from individual precursors. None of the clones developing in vitro from enteric crest-derived cells contain melanocytes, endocrine cells, or skeletal muscle, although some contain smooth muscle. The heterogeneity of the crest-derived cell population that colonizes the bowel is reflected in the birthdays of the different types of enteric neuron (50). Some of these cells, containing acetylcholine or serotonin, are born very early in embryonic life, beginning in the mouse at E8.5, an age that precedes the arrival in the bowel of even the most precocious of enteric neurons. The crest-derived population that arrives in the gut, therefore, must contain a mixture of already-determined postmitotic neurons, as well as the undetermined pluripotent precursors that were revealed by the experiments involving back-transplantation and clonal analysis described above. On the other hand, other types of enteric neuron, such as those that contain calcitonin gene-related peptide (CGRP), do not even begin to leave the mitotic cycle until after the last serotonergic neurons have been born. The fact that the timing of the birthdays of enteric neurons is a function of their phenotypic class was among the earliest observations to suggest that multiple lineages of crest-derived precursors participate in the formation of the ENS. It is at least plausible that neurons in the same lineage might be born at similar times.
Confirmation of the idea that the ENS is formed by multiple lineages of crest-derived precursors came from a study that combined an in vitro investigation of enteric neuronal development with an analysis of the effects of the targeted deletion of the mash-1 transcription factor (51). It had previously been known that cells in the developing mammalian (but not avian) bowel are transiently catecholaminergic (TC) (52-55), and that these cells are derivatives of the neural crest (56-58). The TC cells were found not to be neurons, because they proliferate (56, 57, 59), although it was postulated that the TC cells might be neural precursors. That hypothesis was confirmed, and the disappearance of TC cells was found to occur not because they die, but because they lose their catecholaminergic properties as they acquire the characteristics of their terminally differentiated phenotype (56).
When it was discovered that TC cells express the same cell-surface differentiation antigens as precursors in the sympathoadrenal lineage, it was proposed that enteric and sympathetic neurons arise from a common lineage of crest-derived progenitors (60, 61). This idea was furthered by the demonstration that mash-1 is expressed during the development of both sympathetic and enteric neurons (62, 63). On the other hand, knockout of mash-1 is accompanied by the loss of almost the entire sympathetic nervous system (the superior cervical ganglion is spared), while the development of the ENS (except for that of the esophagus, which is lost) is merely delayed (63, 64( .
The shared features expressed by sympathoadrenal and ENS precursors made it possible to test the hypothesis that sympathetic and enteric neurons are derivatives of a common precursor lineage. Complement-mediated lysis of all of the cells isolated from the bowel that express antigens common to the sympathoadrenal lineage reduces the numbers of enteric neurons developing in vitro, but it does not prevent their formation (51). No serotonergic neurons develop in vitro following lysis of TC cells, although neurons expressing CGRP appear normally. The cells in the developing bowel that express mash-1 were found to be TC cells, and the knockout of mash-1 in mice was demonstrated to result in the complete loss of early-developing serotonergic neurons, while late-born CGRP-containing cells appear on schedule. The apparent delay in timing of the formation of the ENS in mash-1 knockout mice is thus due to the selective loss of the early-developing lineage of enteric neurons. These observations established for the first time that the ENS is, in fact, derived from at least two lineages of crest-derived precursors. One of these, which may be common to the precursors of sympathoadrenal cells, obligatorily expresses mash-1, gives rise to neurons that are born early, and is the origin of all of the serotonergic neurons of the bowel. The other lineage is never catecholaminergic, does not express mash-1, gives rise to neurons that are born late, and is the origin of peptidergic enteric neurons, including those that contain CGRP.
Since the discovery of the role of mash-1 in the development of one lineage of enteric neurons, additional lineages or sublineages of enteric neuronal precursor have been discovered. All of the crest-derived cells that colonize the bowel appear to express p75NTR, the common neurotrophin receptor (44, 56, 65). The cells (from the vagal and sacral regions of the neural crest) evidently also express Ret (66, 67), which must be stimulated by its functional ligand, glial cell line-derived neurotrophic factor (GDNF) (68-70). Actually, in order to stimulate Ret, GDNF has to form a complex with an a-component, GFRa-1 (71-73). If Ret (74, 75), GDNF (69, 76), or GFRa-1 (77) are knocked out, there are no neurons or glia in the entire bowel below the esophagus and in the immediately adjacent region of the stomach. GDNF is a mitogen for early crest-derived enteric neuronal precursors and later supports their development as neurons (78-80). Because the defect in the mash-1 knockout mice is much more limited than that seen after the elimination of Ret or the components of its ligand complex, it follows that the mash-1-dependent lineage is a subset of a larger lineage of GDNF-dependent precursors.
More limited sublineages have been identified by the dependence of subsets of enteric neurons on other growth factor/receptor complexes, such as a still-to-be identified neuropoietic cytokine that activates the alpha component of the receptor for CNTF (65). Other factors, such as NT-3/TrkC (81(and laminin-1 (82, 83), have been identified that promote the development of enteric neurons, but these factors do not define particular lineages. Endothelin-3 (ET-3) and its preferred receptor, endothelin B (ETB), also appear to be necessary for a subset of enteric neurons (84, 85). This factor/receptor complex, however, does not appear to define a particular lineage of enteric neurons. When either ET-3 (84) or ETB (85) is knocked out in mice, the terminal colon becomes aganglionic, just as it does in humans with Hirschsprung's disease, some of whom also display ET-3 (86) or ETB (87) mutations. The lesion that results from deletion of ET-3 or ETB thus is geographically restricted, but within the affected zone all lineages of crest-derived enteric neurons are affected. ET-3 has been found to inhibit enteric neuronal development, and it has been postulated to act by preventing the premature differentiation of enteric neurons before their crest-derived have completed their colonization of the gut (78, 88).
4. Summary
The concept of lineage is used in two ways in describing development. In the pedigree sense, lineage is used to refer to the descendants of a common progenitor. Sharing a lineage defined as a pedigree, however, does not necessarily mean that the descendants of the common progenitor share the same developmental program or fate. Lineage as a pedigree is thus independent of the determination of its members. In a second developmental sense, the term lineage is used to refer to cells that do follow a common developmental program, regardless of whether these cells trace their ancestry back to a single shared precursor. Usually they do not. Identity of determination thus is now the common denominator that defines lineage.
References
1. J. B. L. Bard (1990) Morphogenesis: The Cellular and molecular processes of Developmental Anatomy, Cambridge University Press, Cambridge, U.K.
2. J. P. Trinkaus (1984) Cells into Organs: The Forces That Shape the Embryo, 2nd ed., Prentice-Hall, Engelwood Cliffs, NJ.
3. M. R. Rebagliati et al. (1985) Cell 42, 769–777.
4. J. B. Gurdon (1992) Cell 68, 185–199.
5. J. P. Vincent, G. F. Oster, and J. C. Gerhart (1986) Dev. Biol. 113, 484–500.
6. M. Kirchner, J. Newport, and J. Gerhart (1985) Trends Genet. 1, 41–47.
7. S. C. Guthrie and N. B. Gilula (1989) Trends Neurosci. 12, 12–16.
8. J. D. Hardin and L. Y. Cheng (1986) Dev. Biol. 115, 490–501.
9. H. Spemann (1938) Embryonic Development and Induction, Yale University Press, New Haven, CT.
10. W. C. Smith et al. (1993) Nature 361, 547–549.
11. K. W. Y. Cho et al. (1991) Cell 66, 1111–1120.
12. R. L. Gardner (1985) Philos. Trans. R. Soc. Lond. (Biol.) 312, 163–178.
13. S. J. Kelly (1977) J. Exp. Zool. 200, 365–376.
14. A. K. Tarkowski (1959) Nature 184, 1286–1287.
15. A. McLaren (1976) Mammalian Chimeras, Cambridge University Press, Cambridge, U.K.
16. C. Kenyon (1985) Philos. Trans. R. Soc. Lond. (Biol.) 312, 21–38.
17. J. E. Sulston and H. R. Horvitz (1977) Dev. Biol. 56, 110–156.
18. D. D. Galileo et al. (1990) Proc. Natl. Acad. Sci. USA 87, 458–462.
19. C. Walsh and C. Reid (1995) Ciba Found. Symp. 193, 21–40.
20. J. G. Parnavelas, M. C. Mione, and A. Lavdas (1995) Ciba Found Symp. 193, 41–58.
21. J. E. Sulston, E. Schierenberg, and J. G. White (1983) Dev. Biol. 100, 64–119.
22. B. Christ, H. J. Jacob, and M. Jacob (1977) Anat. Embryol. 150, 171–186.
23. R. McKay (1997) Science 276, 66–71.
24. D. M. Orentas and R. H. Miller (1996) Dev. Biol. 177, 43–53.
25. K. K. Johe et al. (1997) Genes Dev. 10, 3129–3140.
26. R. E. Gross et al. (1996) Neuron 17, 595–606.
27. L. Lillien (1998) Curr. Opin. Neurobiol. 8(1), 37–44.
28. N. M. Le Douarin and E. Dupin (1993) J. Neurobiol. 24, 146–161.
29. D. J. Anderson (1989) Neuron 3, 1–12.
30. N. M. Le Douarin (1982) The Neural Crest, Cambridge University Press, Cambridge, U.K.
31. N. M. Le Douarin (1986) Science 231, 1515–1522.
32. K. Ito, T. Morita, and M. Sieber-Blum (1993) Dev. Biol. 157, 517–525.
33. M. Sieber-Blum and A. M. Cohen (1980) Dev. Biol. 80, 96–106.
34. M. Sieber-Blum et al. (1993) J. Neurobiol. 24(2), 173–184.
35. A. Baroffio, E. Dupin, and N. M. Le Douarin (1988) Proc. Natl. Acad. Sci. USA 85, 5325–5329.
36. M. Bronner-Fraser and S. E. Fraser (1988) Nature 335, 161–164.
37. M. Bronner-Fraser and S. Fraser (1989) Neuron 3, 755–766.
38. S. E. Fraser and M. Bronner-Fraser (1991) Development 112, 913–920.
39. D. L. Stemple and D. J. Anderson (1993) Dev. Biol. 159, 12–23.
40. D. Stemple and D. J. Anderson (1992) Cell 71, 973–985.
41. P. D. Henion and J. A. Weston (1997) Development 124(21), 4351–4359.
42. C. A. Erickson and T. L. Goins (1995) Development 121, 915–924.
43. D. J. Anderson (1993) Annu. Rev. Neurosci. 16, 129–158.
44. M. D. Gershon (1997) Curr. Opin. Neurobiol. 7, 101–109.
45. T. P. Rothman et al. (1990) Development 109, 411–423.
46. T. P. Rothman et al. (1993) Dev. Dyn. 196, 217–233.
47. F. Sextier-Sainte-Claire Deville, C. Ziller, and N. M. Le Douarin (1994) Dev. Biol. 163, 141–151.
48. L. Lo and D. J. Anderson (1995) Neuron 15(3), 527–539.
49. L. Lo, L. Sommer, and D. J. Anderson (1997) Curr. Biol. 7, 440–450.
50. T. D. Pham, M. D. Gershon, and T. P. Rothman (1991) J. Comp. Neurol. 314, 789–798.
51. E. Blaugrund et al. (1996) Development 122, 309–320.
52. P. Cochard, M. Goldstein, and I. B. Black (1978) Proc. Natl. Acad. Sci. USA 75, 2986–2990.
53. G. M. Jonakait et al. (1979) Proc. Natl. Acad. Sci. USA 76, 4683–4686.
54. G. Teitelman, T. H. Joh, and D. J. Reis (1978) Brain Res. 158, 229–234.
55. M. D. Gershon et al. (1984) J. Neurosci. 4, 2269–2280.
56. G. Baetge, J. E. Pintar, and M. D. Gershon (1990) Dev. Biol. 141, 353–380.
57. G. Baetge and M. D. Gershon (1989) Dev. Biol. 132, 189–211.
58. G. Baetge, K. A. Schneider, and M. D. Gershon (1990) Development 110, 689–701.
59. G. Teitelman et al. (1981) Dev. Biol. 86, 348–355.
60. D. J. Anderson et al. (1991) J. Neurosci. 11, 3507–3519.
61. J. F. Carnahan, D. J. Anderson, and P. H. Patterson (1991) Dev. Biol. 148, 552–561.
62. F. Guillemot and A. L. Joyner (1993) Mech. Dev. 42, 171–185.
63. F. Guillemot et al. (1993) Cell 75, 463–476.
64. L. Lo et al. (1994) Perspect. Dev. Neurobiol. 2, 191–201.
65. A. Chalazonitis et al. (1998) Dev. Biol. 198(2), 343–365.
66. V. Pachnis, B. Mankoo, and F. Costantini (1993) Development 119, 1005–1017.
67. P. L. Durbec et al. (1996) Development 122, 349–358
68. P. Durbec et al. (1996) Nature 381–793.
69. S. Jing et al. (1996) Cell 85, 1113–1124.
70. M. Trupp et al. (1996) Nature 381, 785–789.
71. M. Trupp et al. (1997) J. Neurosci. 17, 3554–3567.
72. A. M. Davies et al. (1997) Neuron 19, 485.
73. J. Widenfalk et al. (1997) J. Neurosci. 17, 8506–8519.
74. A. Schuchardt et al. (1994) Nature 367, 380–383.
75. J. J. S. Treanor et al. (1996) Nature 382, 80–83.
76. M. Sénchez et al. (1996) Nature 382, 70–73.
77. G. Cacalano et al. (1998) Neuron 21, 53–62.
78. C. J. Hearn, M. Murphy, and D. Newgreen (1998) Dev. Biol. 197, 93–105.
79. R. O. Heuckeroth et al. (1998) Dev. Biol. 200(1), 116–129.
80. A. Chalazonitis et al. (1998) Dev. Biol. 204, 385–406.
81. A. Chalazonitis et al. (1994) J. Neurosci. 14, 6571–6584.
82. T. P. Rothman et al. (1996) Dev. Biol. 178, 498–513.
83. A. Chalazonitis et al. (1997) J. Neurobiol. 33, 118–138.
84. A. G. Baynash et al. (1994) Cell 79, 1277–1285.
85. K. Hosoda et al. (1994) Cell 79, 1267–1276.
86. P. Edery et al. (1996) Nat. Genet. 12(4), 442–444.
87. E. G. Puffenberger et al. (1994) Cell 79, 1257–1266.
88. J. J. Wu, T. P. Rothman, and M. D. Gershon (1997) Neurosci. Abst. 23, 24.
الاكثر قراءة في مواضيع عامة في الاحياء الجزيئي
اخر الاخبار
اخبار العتبة العباسية المقدسة
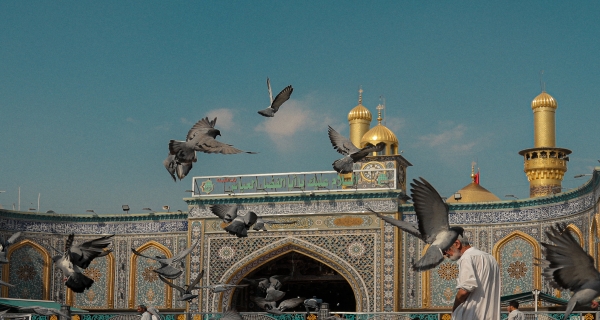
الآخبار الصحية
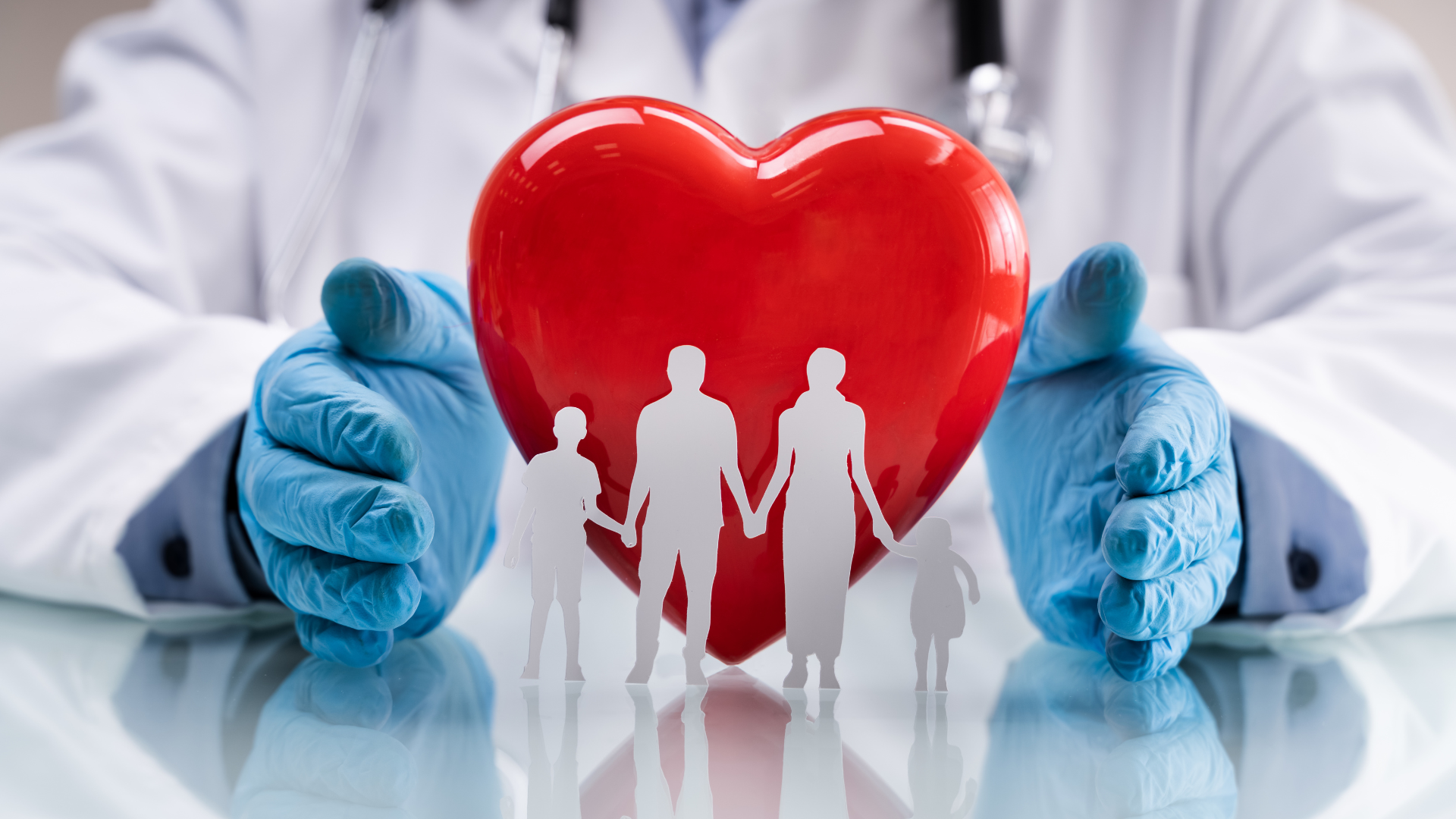