النبات
مواضيع عامة في علم النبات
الجذور - السيقان - الأوراق
النباتات الوعائية واللاوعائية
البذور (مغطاة البذور - عاريات البذور)
الطحالب
النباتات الطبية
الحيوان
مواضيع عامة في علم الحيوان
علم التشريح
التنوع الإحيائي
البايلوجيا الخلوية
الأحياء المجهرية
البكتيريا
الفطريات
الطفيليات
الفايروسات
علم الأمراض
الاورام
الامراض الوراثية
الامراض المناعية
الامراض المدارية
اضطرابات الدورة الدموية
مواضيع عامة في علم الامراض
الحشرات
التقانة الإحيائية
مواضيع عامة في التقانة الإحيائية
التقنية الحيوية المكروبية
التقنية الحيوية والميكروبات
الفعاليات الحيوية
وراثة الاحياء المجهرية
تصنيف الاحياء المجهرية
الاحياء المجهرية في الطبيعة
أيض الاجهاد
التقنية الحيوية والبيئة
التقنية الحيوية والطب
التقنية الحيوية والزراعة
التقنية الحيوية والصناعة
التقنية الحيوية والطاقة
البحار والطحالب الصغيرة
عزل البروتين
هندسة الجينات
التقنية الحياتية النانوية
مفاهيم التقنية الحيوية النانوية
التراكيب النانوية والمجاهر المستخدمة في رؤيتها
تصنيع وتخليق المواد النانوية
تطبيقات التقنية النانوية والحيوية النانوية
الرقائق والمتحسسات الحيوية
المصفوفات المجهرية وحاسوب الدنا
اللقاحات
البيئة والتلوث
علم الأجنة
اعضاء التكاثر وتشكل الاعراس
الاخصاب
التشطر
العصيبة وتشكل الجسيدات
تشكل اللواحق الجنينية
تكون المعيدة وظهور الطبقات الجنينية
مقدمة لعلم الاجنة
الأحياء الجزيئي
مواضيع عامة في الاحياء الجزيئي
علم وظائف الأعضاء
الغدد
مواضيع عامة في الغدد
الغدد الصم و هرموناتها
الجسم تحت السريري
الغدة النخامية
الغدة الكظرية
الغدة التناسلية
الغدة الدرقية والجار الدرقية
الغدة البنكرياسية
الغدة الصنوبرية
مواضيع عامة في علم وظائف الاعضاء
الخلية الحيوانية
الجهاز العصبي
أعضاء الحس
الجهاز العضلي
السوائل الجسمية
الجهاز الدوري والليمف
الجهاز التنفسي
الجهاز الهضمي
الجهاز البولي
المضادات الحيوية
مواضيع عامة في المضادات الحيوية
مضادات البكتيريا
مضادات الفطريات
مضادات الطفيليات
مضادات الفايروسات
علم الخلية
الوراثة
الأحياء العامة
المناعة
التحليلات المرضية
الكيمياء الحيوية
مواضيع متنوعة أخرى
الانزيمات
Bohr Effect
المؤلف:
M. F. Perutz, G. Fermi, C. Poyart, J. Pagnier, and J. Kister
المصدر:
J. Mol. Biol. 233, 536–545
الجزء والصفحة:
14-12-2015
2884
Bohr Effect
Bohr et al. (1) discovered that the oxygen dissociation curve of blood shifts to higher partial pressures of oxygen in the presence of increasing concentrations of carbon dioxide by lowering the oxygen affinity of hemoglobin. This effect, it was concluded, results from the concomitant decrease in pH, and the effect of pH on the oxygen affinity of hemoglobin has subsequently been known as the Bohr effect. It was subsequently discovered that CO2 also lowers the oxygen affinity of hemoglobin (Hb) directly by binding to its a-amino groups, forming carbamino compounds (2, 3:(
The original observations of Bohr et al. (1) are now known as the “classical Bohr effect,” which is a composite of the specific effects of H+ and CO2.
The CO2 content of blood is reduced upon oxygenation of hemoglobin at a constant CO2 pressure (4). This phenomenon, known as the “classical Haldane effect,” is a combination of the oxygen-linked dissociation of the carbamino groups (Eq. 1) and release of protons
followed by dehydration of bicarbonate:
The release of protons upon oxygenation or their uptake upon deoxygenation of hemoglobin is known as the “Haldane effect.”
The Bohr effect and the Haldane effect are thermodynamically equivalent (5) because they are linked functions (6). Whatever effect oxygen binding has on the affinity of hemoglobin for protons, changes in the pH must have the same effect on the affinity of hemoglobin for oxygen:
where H+ is the number of protons bound per heme group, Y is the fraction of hemoglobin binding sites occupied by O2, and PO2 is the partial pressure of O2. The left-hand side of Eq. 4 is known as the “Haldane coefficient,” and the right-hand side is the “Bohr coefficient.” They give the magnitude of the Haldane and Bohr effects, which must be the same.
These effects are physiologically important for efficient and regulated transport of O2 and CO2 in opposite directions (see Hemoglobin). When the shape of the oxygen dissociation curve expressed by Y versus log PO2 is independent of changes in pH, at least within the range 0.1 < Y < 0.9, Eq. 4 is simplified to
where P50 is the PO2 at Y = 0.5 and d is the Bohr coefficient, which is independent of the degree of O2 binding. The Bohr coefficient expresses the number of protons (per heme group) bound to hemoglobin upon full oxygenation and is the same as d of Eq. 2.
d is negative above pH 6.3, and protons are released upon oxygenation of hemoglobin, whereas d is positive at lower pH values, and protons are taken up upon oxygenation. These two phenomena are known respectively as the “alkaline” and “acid” Bohr effects. At physiological pH 7.4, human adult hemoglobin A (HbA) has d = –0.6 in the presence of 0.1 M NaCl. This value is halved in the absence of Cl– .
The Bohr effect arises from changes in the pKa values of particular ionizable groups of hemoglobin as a result of changes in their environment upon binding oxygen. The group primarily responsible for the alkaline Bohr effect of human HbA is the imidazole side chain of the C-terminal His146 of the b chain. Its pKa value changes from 8.0 to 7.1 upon oxygenation, contributing the greater part of the alkaline Bohr effect in the absence of Cl–. In the presence of Cl–, binding of these ions to several ionizable groups that line the central cavity of the hemoglobin molecule, including the a-amino groups of the a chains and the -amino groups of Lys82 of the b chains, affects the protonation of those groups. Consequently, the release of Cl– ions upon binding of oxygen produces an additional, Cl– -dependent Bohr effect (7). It is thought that His143 of the b chains is one of the groups responsible for the acid Bohr effect (8), but the others are still unidentified.
The term “Bohr effect” is sometimes used for pH-dependent binding of other ligands, such as CO2 and alkylisocyanides, to hemoglobin. It is also used for pH-dependent ligand binding to oxygen-binding proteins other than hemoglobin.
References
1.C. Bohr, K. A. Hasselbalch, and A. Krogh (1904) Skand. Arch. Physiol. 16, 402–412.
2.J. K. W. Ferguson and F. J. W. Roughton (1934) J. Physiol. (London) 83, 87–102.
3.J. V. Kilmartin and L. Rossi-Bernardi (1969) Nature 222, 1243–1246.
4.J. Christiansen, C. C. Douglas, and J. S. Haldane (1914) J. Physiol. (London) 48, 244–277.
5.I. Tyuma and Y. Ueda (1975) Biochem. Biophys. Res. Commun. 65, 1278–1283.
6.J. Wyman (1964) Adv. Protein Chem. 19, 223–286.
7. M. F. Perutz, G. Fermi, C. Poyart, J. Pagnier, and J. Kister (1993) J. Mol. Biol. 233, 536–545.
8.M. F. Perutz, J. V. Kilmartin, K. Nishikura, J. H. Fogg, P. J. G. Butler, and H. S. Rollema (1980) J. Mol. Biol. 138, 649–670.
الاكثر قراءة في مواضيع عامة في الاحياء الجزيئي
اخر الاخبار
اخبار العتبة العباسية المقدسة
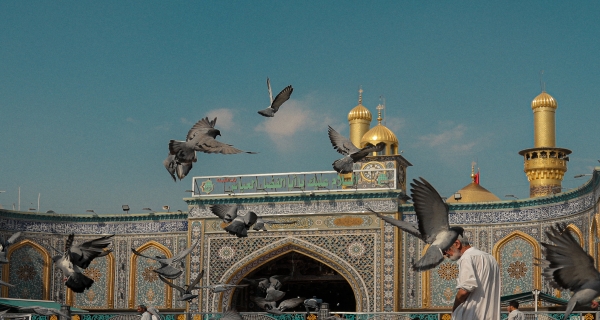
الآخبار الصحية
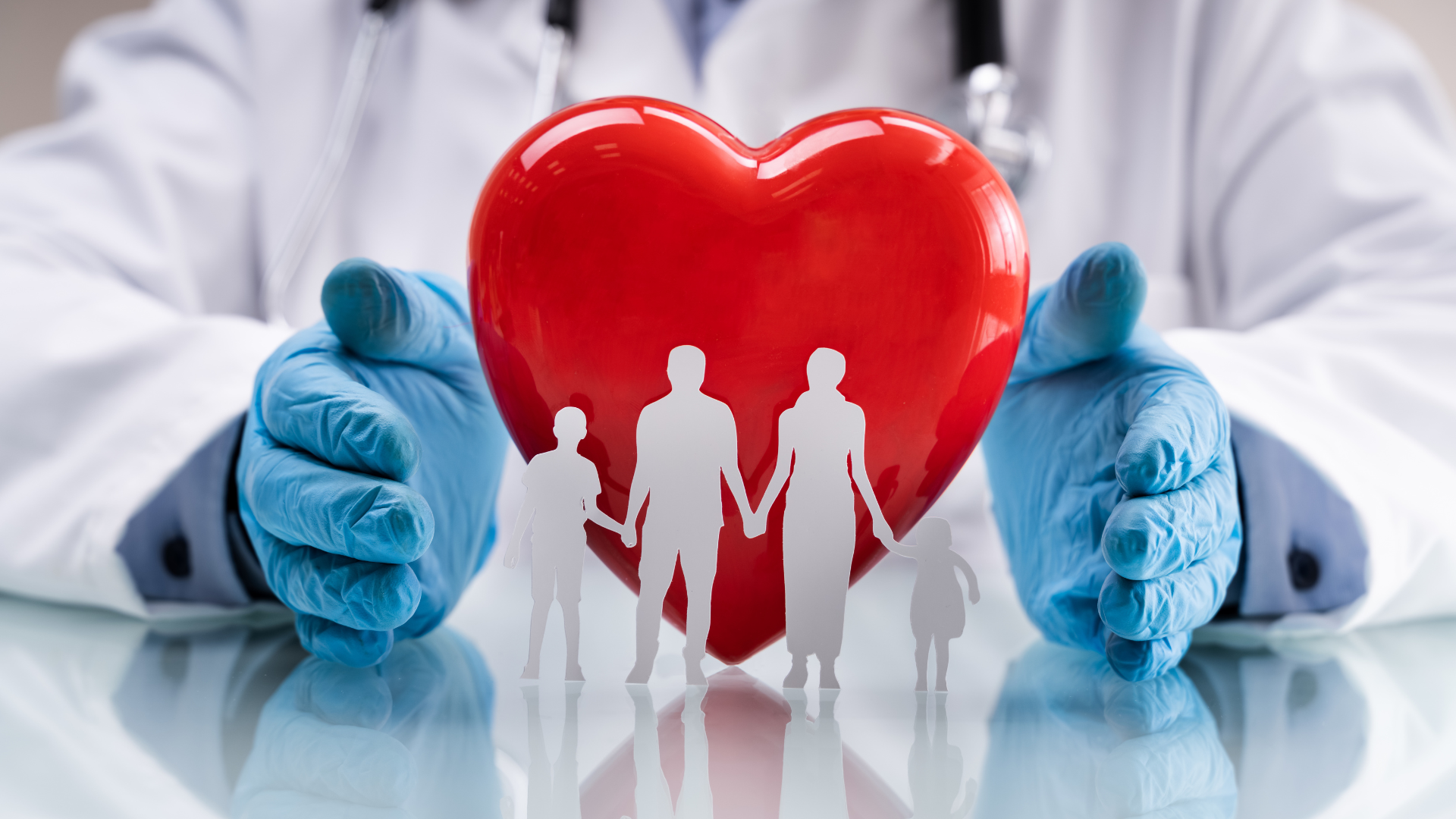