النبات
مواضيع عامة في علم النبات
الجذور - السيقان - الأوراق
النباتات الوعائية واللاوعائية
البذور (مغطاة البذور - عاريات البذور)
الطحالب
النباتات الطبية
الحيوان
مواضيع عامة في علم الحيوان
علم التشريح
التنوع الإحيائي
البايلوجيا الخلوية
الأحياء المجهرية
البكتيريا
الفطريات
الطفيليات
الفايروسات
علم الأمراض
الاورام
الامراض الوراثية
الامراض المناعية
الامراض المدارية
اضطرابات الدورة الدموية
مواضيع عامة في علم الامراض
الحشرات
التقانة الإحيائية
مواضيع عامة في التقانة الإحيائية
التقنية الحيوية المكروبية
التقنية الحيوية والميكروبات
الفعاليات الحيوية
وراثة الاحياء المجهرية
تصنيف الاحياء المجهرية
الاحياء المجهرية في الطبيعة
أيض الاجهاد
التقنية الحيوية والبيئة
التقنية الحيوية والطب
التقنية الحيوية والزراعة
التقنية الحيوية والصناعة
التقنية الحيوية والطاقة
البحار والطحالب الصغيرة
عزل البروتين
هندسة الجينات
التقنية الحياتية النانوية
مفاهيم التقنية الحيوية النانوية
التراكيب النانوية والمجاهر المستخدمة في رؤيتها
تصنيع وتخليق المواد النانوية
تطبيقات التقنية النانوية والحيوية النانوية
الرقائق والمتحسسات الحيوية
المصفوفات المجهرية وحاسوب الدنا
اللقاحات
البيئة والتلوث
علم الأجنة
اعضاء التكاثر وتشكل الاعراس
الاخصاب
التشطر
العصيبة وتشكل الجسيدات
تشكل اللواحق الجنينية
تكون المعيدة وظهور الطبقات الجنينية
مقدمة لعلم الاجنة
الأحياء الجزيئي
مواضيع عامة في الاحياء الجزيئي
علم وظائف الأعضاء
الغدد
مواضيع عامة في الغدد
الغدد الصم و هرموناتها
الجسم تحت السريري
الغدة النخامية
الغدة الكظرية
الغدة التناسلية
الغدة الدرقية والجار الدرقية
الغدة البنكرياسية
الغدة الصنوبرية
مواضيع عامة في علم وظائف الاعضاء
الخلية الحيوانية
الجهاز العصبي
أعضاء الحس
الجهاز العضلي
السوائل الجسمية
الجهاز الدوري والليمف
الجهاز التنفسي
الجهاز الهضمي
الجهاز البولي
المضادات الحيوية
مواضيع عامة في المضادات الحيوية
مضادات البكتيريا
مضادات الفطريات
مضادات الطفيليات
مضادات الفايروسات
علم الخلية
الوراثة
الأحياء العامة
المناعة
التحليلات المرضية
الكيمياء الحيوية
مواضيع متنوعة أخرى
الانزيمات
Beta-Galactosidase of Escherichia Coli
المؤلف:
W. A. Beta-Galactosidase of Escherichia Coli Mohler and H. M. Blau
المصدر:
Proc. Natl. Acad. Sci. USA 93, 12423–12427
الجزء والصفحة:
10-12-2015
4365
Beta-Galactosidase of Escherichia Coli
Exploration of the lactose (lac) system of Escherichia coli was started in the late 40s at the Pasteur Institute in Paris by Jacques Monod and his collaborators. As a result, most of the major concepts of gene expression and regulation were established with this system. The major gene product of the lac operon is b-galactosidase, the enzyme that splits lactose into glucose and galactose and the product of the lacZ gene. In addition to its importance for studies of enzyme induction, this protein has become very useful as a reporter gene in very many studies of gene expression.
1.Characterization
Because of its importance in genetic studies of lac operon, E. coli b-galactosidase was purified and characterized by several groups in the early 50s (9, 10). It could be easily measured quantitatively because of a chromogenic substrate, o-nitrophenyl-b-D-galactoside (ONPG). The enzyme is a hydrolytic transglucosidase and accounts for up to 5% of the total protein in haploid, fully induced or constitutive strains of E. coli. The purified enzyme was crystallized, although its three-dimensional structure had to wait 40 years to be solved. Several procedures for producing large amounts of enzyme have been described (reviewed in Ref. 6). b-galactosidase is a particularly stable enzyme. It can be stored in 40% ammonium sulfate for several years at 4 °C without significant loss of activity. In buffered solution, the enzyme is stable at 37 °C for several weeks. At 57 °C the half-life of heat inactivation is 10 min (11). Active enzyme is recovered with 100% yield after denaturation with 8 M urea, and fairly good recovery is possible after treatment by 6 M guanidinium chloride (12).
b-Galactosidase is specific for the b-D-galactopiranoside configuration and cleaves the bond between the anomeric carbon (C1) and the glycosyl oxygen (10). The enzyme acts as a hydrolase, but also as a transferase. The galactosyl moiety can be transferred to monosaccharides, oligosaccharides, alkyl alcohols, and phenols and also to mercaptoethanol. b-Galactosidase also transfers the galactosyl moiety from the C4 position to the C6 position of glucose to form allolactose, which is the natural inducer of the lac operon (13). b-Galactosidase requires Mg2+ and Na+ ions for maximal activity (9), but the reason for needing these cations is still unclear.
That b-galactosidase is a tetramer of four identical subunits, possessing one active site per subunit, is well documented, and the tetramer is the only active form of the enzyme. Because of its high turnover number (about 6000 moles of ONPG hydrolyzed/sec/mole of enzyme, one molecule of b-galactosidase per bacterial cell can be accurately measured. Several residues (Glu 461, Met 502, Tyr 503, Glu 537) have been identified as important for catalytic function or to be near the active site (14, 15.These residues are found in the three-dimensional structure in close proximity to one another and form a pocket likely to be the substrate-binding site (16).
The amino acid sequence of b-galactosidase, determined before DNA sequencing techniques had been developed, was the longest protein sequence ever determined (17). According to this sequence, the protein contains 1021 amino acid residues and a subunit molecular weight of 116,248. Subsequently, the nucleotide sequence of the lacZ gene was also determined (18), from which it was predicted that b-galactosidase consists of 1023 residues, with a molecular weight of 116,353 per subunit. It is quite remarkable that the amino acid sequence deduced from the DNA sequence differs only in ten amino acid residues from that obtained by amino acid sequencing of such a large protein.
There is also a homologue of b-galactosidase, evolved b-galactosidase (EBG). Normally E. coli does not grow on lactose when the lacZ gene is deleted. However, cells with lacZ deletions were selected that grow on lactose and produce a new b-galactosidase, called EBG (19). The ebgA gene maps at 66 min on the E. coli chromosome, whereas that for lacZ maps at 8 min. The active EBG protein is a hexamer containing 964 residues per monomer. The sequence identity between the ebgA and lacZ genes at the DNA nucleotide level (50%) exceeds that at the amino acid level (30%). This led to the conclusion that the two genes descended by divergence from a common ancestral gene. b-galactosidase and EBG do not cross-react with antisera prepared against each type of protein.
2.b-Galactosidase Complementation
The term complementation has been generally used in genetics for the phenomenon by which a biological function lost or altered by a mutation is restored through mutual compensation by two differently altered mutant genes. In the case of intracistronic complementation, where the two mutations occur within different copies of the same cistron, it is generally accepted that the repair of function occurs at the level of the protein product of the gene and involves the interaction of differently altered polypeptide chains. Such complementation involves noncovalent interaction of polypeptide chains and may occur by reassociation of differently altered subunits of an oligomeric protein or by interaction between fragments of a single polypeptide chain. Studies of b-galactosidase complementation contributed to elucidating the structure of the enzyme and to understanding the mechanism of the specific recognition, reassociation, and folding of proteins (for reviews, see Refs. 20, 21).
2.1. Complementation Between Point Mutants
Jacob and Monod (8) showed that heterogenotes carrying different lacZ– point mutants may become lacZ+ by complementation. In vitro studies carried out by Perrin (22) showed that many of the inactive proteins containing these point mutations exhibit monomeric structures, but cross-react immunologically with b-galactosidase. In contrast, the active, complemented, b-galactosidase had a sedimentation coefficient like tetrameric wild-type 16 S, although it was more heat-labile. The restoration of enzyme activity in this type of complementation can be accounted for by a mechanism suggested by Crick and Orgel (23), repair of lesions by reassociation of differently altered subunits.
Some inactive b-galactosidase proteins with point mutations exist as dimers. A specific class of these mutants can be activated up to 1000 times by specific antibodies raised against wild-type b-galactosidase (24, 25). The activating antibody directs the formation of active tetramers. In a strict sense this is not a complementation reaction, but the kinetics of activation are similar to those of complementation between point mutant proteins in vitro: In both cases, the appearance of b-galactosidase activity is a relatively slow process, requiring about 300 min at 37 °C.
2.2. Complementation Between Deletion Mutants
The isolation of deletion mutants of the lacZ gene involving quite large genetic segments (26) led to the discovery of a new type of intracistronic complementation (for a review, see Ref. 27). Inactive lacZ deletion mutants, lacking part of the gene corresponding to either the amino- or the carboxy-terminal region of the b-galactosidase polypeptide chain, would complement another inactive deletion mutant containing the region missing in the first. The N- and C-terminal regions involved in the two distinct classes of complementation are known as the a and w regions, respectively (Fig. 1).
Both a- and w-complementation represent noncovalent reassociation of complementary fragments of the b-galactosidase subunit polypeptide chain, which then can reassemble into an enzymatically active tetrameric structure. a- and w-complementation takes place in vivo in partial diploids and in vitro, with extracts of relevant mutant strains.
Figure 1. Diagrammatic representation of the lacZ gene. The figures and letters above the solid line indicate the position of point mutations in the lacZ gene: o, operator; y, structural gene of Lac permease. The figures below the line represent recombination frequencies, as determined by crosses between two point mutants. The lines below indicate the extent of various deletions: M 15 isolated by Beckwith (28); A 238 and W 4680, isolated by Cook and Lederberg (29); all others by Jacob, Ullmann and Monod (26). Reprinted from (20) by permission of Cold Spring Harbor Laboratory.
2.3. a-Complementation
All mutants that have their promoter-proximal segment (a-segment) intact are a-donors. All genes that contain partial deletions of the N-terminus not extending beyond a barrier (which is located by the dashed line in Figure 1 between mutants 274 and X64) are a-acceptors when the proteins they produce are mixed with that of an a-donor (30). The time course of in vitro a-complementation is relatively slow and reaches a plateau in about 3 h. The a-complemented enzyme, like wild-type b-galactosidase, is a tetramer but is less stable to heat or urea treatment. The equilibrium constant for the complementation reaction was estimated to be approximately 1 to 2 × 109M–1 (21). Given the slow association rate constant, one can predict a very low dissociation rate, indicating that the complementation process is virtually irreversible under physiological conditions.
The most unusual property of the a-peptide is its high temperature resistance in 6 M guanidinium chloride. Bacterial extracts of lacZ strains containing a-donors liberate soluble a-peptides after boiling in this denaturant (30) or after autoclaving in its absence (31). The smallest a-peptide still capable of complementation has a size of about 7400 Da. Upon cleavage of b-galactosidase with cyanogen bromide, Langley et al. (32) isolated and sequenced a peptide that has a-donor activity corresponding to residues 3 to 92 of the polypeptide chain.
The best studied a-acceptor is the M15 protein, produced by the lacZ mutant DM15 isolated by Beckwith (28) and shown genetically to be a small deletion in the early part of the gene (Fig. 1). The M15 protein lacks residues 11 through 41 of b-galactosidase and is a dimer (33). It has a trace level of enzyme activity and can be significantly activated by addition of anti-b-galactosidase antibodies (34), up to 15% of that obtained by a-complementation.
The nature of the a-complementation process and its probable pathway have been studied extensively (21). It is believed that the a-peptide modifies the quaternary structure of the a-acceptor by causing its tetramerization and consequently the recovery of enzymatic activity. The three-dimensional structure of b-galactosidase (16) fully confirms this prediction by showing that the a-peptide segment of the polypeptide chain participates in forming specific subunit contacts.
2.4. w-Complementation
All mutants that have their promoter-distal region (w-region) intact are w-donors. They complement w-acceptors that are products of deletion or point mutants in the w-region (27) (Fig. 1), which represents about one-third of the total genetic length of lacZ. The purified w-peptide has a molecular weight of 40,000 Da (35). The kinetics of w-complementation are faster than those of a-complementation and reach a maximum extent in about 1 h. Although w-peptide is reversibly renatured from 8 M urea or 6 M guanidinium chloride, it is highly heat-labile.
w-Acceptor proteins active in complementation have not been obtained in pure form. In crude bacterial extracts (36, 37), w-acceptor exists in different forms of aggregation, depending on the ionic strength. At low ionic strength it is probably a tetramer that dissociates at high ionic strength, most probably into monomers. Both forms are active in complementation. The w-complemented enzyme is a tetramer, like the wild type, and exhibits very similar catalytic properties. The main difference appears after denaturation in 8 M urea. Recovery of its enzyme activity after renaturation does not exceed 5%, whereas it is practically 100% with wild-type enzyme. Moreover, the w-peptide is recovered quantitatively from the complemented enzyme after urea treatment (11).
One of the most remarkable features of w-complementation is the high affinity and specificity that the peptide fragments exhibit. They reassociate even at high dilution and in the presence of a large excess of foreign proteins. The yield of complementation is considerably increased in the presence of a b-galactosidase substrate analog or in the presence of anti-b-galactosidase antibodies (36, 37). Comparing wild-type and w-complemented b-galactosidase, Goldberg (38) proposed a model for the tertiary structure of the two monomers. After having shown that the active, complemented enzyme involves reassociation into a tetrameric structure of four w-acceptor polypeptides with four w-peptides, he showed that both w and acceptor are folded to form compact globular structures, close to those of the corresponding segments of the complete polypeptide within the native enzyme. This “globule model” involves the notion that large polypeptide chains contain several distinct domains that assemble specifically to give the polypeptide chain its final tertiary structure. The well-defined compact w-domain is apparent within the native monomer in the three-dimensional structure of b-galactosidase (16), which also shows an unstructured 10-residue exposed segment that joins the w-domain to the rest of the chain. This explains, a posteriori, why mild proteolytic treatment of the wild-type b-galactosidase liberates significant amounts of w-peptide (11).
3.Three-Dimensional Structure
The structure of tetrameric b-galactosidase was solved by X-ray crystallography at 2.5 Å resolution by B.W. Matthews et al. (16). It is the longest polypeptide chain for which an atomic structure has been determined. The crystal structure shows that b-galactosidase is a tetramer with dimensions of roughly 175 × 135 × 90 Å along the respective twofold axes. The constituent monomers interact through two different monomer–monomer contacts. The b-galactosidase monomer consists of five compact domains and a relatively extended 50-residue N-terminal segment, corresponding to the a-peptide, which contributes to the activating interface (Fig. 2). The monomer is relatively flat and elongated and has approximate dimensions of 50 × 100 × 40 Å. The first domain consists of residues 52 to 217, the second of residues 220 to 334, the third of residues 334 to 627, the fourth of residues 627 to 736, and the final fifth domain, corresponding to the w peptide, of residues 737 to 1023. Topologically, the second domain is identical to the fourth domain. The modular structure of the monomer corroborates earlier findings (37, 38), which suggested that the folding of this rather long polypeptide chain is facilitated by each domain acting as an independent unit.
Figure 2. Three-dimensional structure of b-galactosidase. (a) Ribbon representation of the b-galactosidase tetramer showing the largest face of the molecule. Contacts between red/green and blue/yellow dimers form the long interface. Contacts between the red/yellow and blue/green dimers form the activating interface. Formation of the tetrameric particle results in two deep clefts that run across opposite faces of the molecule. Each contains two active sites. (b(Ribbon diagram of the blue/green dimer viewed down the molecular two-fold axis, showing the composition of the activating interface. Residues 1 to 50 from each chain, which form the a-complementation region, are shown in red. The interface includes contacts between the respective complementation peptides, between two a-helices from the respective monomers that pack together to form a four-helix bundle and between an extended loop (residues 272 to 288) from each monomer that reaches across the interface and extends into the active-site region of the neighboring monomer, stabilizing the active site structure. (c) Stereo ribbon diagram of the b-galactosidase monomer showing the domain organization of the chain. Residues corresponding to successive domains are colored in successive spectral colors. See color insert.
At present, no structure of a complex of b-galactosidase with a substrate or analog is available, so the specific interaction sites between enzyme and substrate are not known. Nevertheless, earlier biochemical studies (14, 15) concluded that residues important for catalytic function or near the active site (Glu461, Met502, Tyr503, Glu537) are found close to one another and located around a deep pit within the third domain, which is postulated to be the substrate-binding site. Residues from distant regions of the sequence (Trp568, Trp999) also contribute to the active site.
The X-ray structure of b-galactosidase provides a structural rationale for both a- and w-complementation. The N-terminal segment, representing the a peptide, participates in forming a subunit interface, which in turn allows formation of the active site, made up of elements from two different subunits. The w-fragment, corresponding to the fifth domain of the subunit, exhibits a specific topology. Rotation of this domain by more than 90° positions Trp 999 at the active site in the vicinity of the third domain.
4.b-Galactosidase as a Tool
The b-galactosidase of E. coli is one of the most commonly used enzymes in molecular biology for several reasons: (1) numerous genetic and biochemical aspects of the lac system are known; (2(several indicator media are available for detecting lactose metabolism and monitoring Lac+ and Lac–bacteria; (3) the assay procedure based on o-nitrophenyl-b-D-galactoside (ONPG) hydrolysis is exquisitely sensitive; (4) the protein is highly stable and easy to purify; and (5) the availability of substituted galactoside derivatives permits selecting and screening lac mutants exhibiting a variety of phenotypes. For example, the chromogenic noninducing and colorless substrate, 5-bromo-4-chloro-3-indolyl-b-D-galactoside (X-Gal), forms an insoluble blue dye upon hydrolysis, thus providing a sensitive test for b-galactosidase in solid media. X-Gal also discriminates between strains that produce high and low levels of b-galactosidase, allowing detection of plaque-forming bacteriophages that carry the lacZ gene.
5. b-Galactosidase Fusions
The observation that the N-terminal 23 residues of b-galactosidase can be replaced with other amino acids without affecting enzymatic activity enabled Müller-Hill and Kania (39) to obtain fusions between lacI and lacZ genes that produce a hybrid protein that has both Lac repressor and b-galactosidase activities. Several genetic methods have been developed to construct lac fusions that produce hybrid genes which specify hybrid proteins. Subsequently, in vitro construction of hybrid genes facilitated obtaining a variety of fusions (40). A number of commercially available vectors exist for constructing lacZ fusions in vitro. They contain a lacZ gene truncated at the 5′-end and preceded by a synthetic oligonucleotide containing multiple restriction enzyme cleavage sites, a polycloning site. Thus, if a 5′-coding sequence is cloned into one of these sites so that transcription and translation are restored across lacZ, a hybrid protein with b-galactosidase activity is produced. The sensitive detection of this activity with X-Gal has made b-galactosidase one of the most commonly used in vivo reporter enzymes. Using the lacZ gene as a reporter provides a sensitive method to detect genes subject to specific regulatory signals or to study the mechanism localizing a protein to a given cellular compartment. The regulation, identification, and localization of many proteins of various origins have been uncovered by using the b-galactosidase activity of fusion proteins as a marker.
During the last few years, a number of new eukaryotic expression vectors that produce b-galactosidase fusion proteins have been designed. They are currently being used to analyze developmentally regulated genes, transgenic expression, tissue-specific expression, and a number of other aspects involved in embryogenesis or developmental biology. This approach has been facilitated by using replication-defective retroviral vectors that encode the lacZ gene (41), which has become a widely used cell lineage marking system. The labeled cells are easily identified by histochemical staining, using X-Gal or immunofluorescence with antibodies raised against b-galactosidase.
b-galactosidase fusions provide a unique and versatile experimental tool. The hybrid proteins are easy to purify (42) and can be used as antigen to raise antibodies against the target gene product. In addition, because there is no restriction on the size of the target gene DNA fused to lacZ and because many hybrid proteins retain all or a portion of the activity of the target gene product, these fusions provide a means to identify functional domains within the target gene product.
5.1.a-Complementation
Perhaps the most widely exploited property of b-galactosidase is a-complementation, described previously. Whereas the region of the foreign protein that can replace the N-terminus of b-galactosidase is more or less random in hybrid fusion proteins, replacements in the a-peptide for a-complementation are more restrictive. That insertion of a few amino acid residues does not always interfere with complementation capacity (43) was widely used during the late 70s to develop new cloning vectors, now commercially available. Indeed, a-complementation has become one of the most commonly used methods for identifying bacterial colonies containing recombinant DNA.
The first vector based on a-complementation was developed by Messing et al. (44). Within the major intergenic region of the filamentous bacteriophage M13, they inserted the lac regulatory region and the DNA sequence that codes for the first 146 amino acids of b-galactosidase that have a-complementation capacity. When infected with these phages, bacteria that produce the M15 a-acceptor protein produce active b-galactosidase by complementation and form blue plaques in the presence of the chromogenic substrate X-Gal. Insertion of foreign DNA into the a region of M13 interferes with a-complementation and gives rise to recombinants that form pale blue or colorless plaques. This simple test has made cloning in filamentous bacteriophages a routine procedure. The insertion of a series of synthetic restriction enzyme sites into the a-region provides a variety of targets for cloning foreign DNA fragments. Insertion of these polycloning sites, representing up to 18 extra amino acid residues, into the a-region has practically no effect on a-complementation capacity. Insertion of additional DNA into a cloning site, however, generally abolishes a-complementation and creates recombinant bacteriophages that produce colorless plaques when grown on X-Gal plates (blue/white screen). The great advantage of using vectors generated from single-stranded DNA phage, like M13, is that one obtains both the double-stranded replicative form from the infected bacteria and the single-stranded DNA, from the progeny phage. The latter is frequently used as a template for sequencing with the dideoxy-mediated chain-terminating Sanger method (45) and also for in vitro mutagenesis. Many other vectors that permit identification of recombinant clones based on a-complementation have been designed subsequently. The most commonly used are the pUC vectors (46).
Recently, Mohler and Blau (47) adapted intracistronic complementation of the lacZ gene for use in eukaryotic cells. By constructing replication-defective retroviral vectors that express a-donors and a-acceptors and w-donors and w-acceptors, complementation of the relevant lacZ mutants in mammalian cells permits analyzing cell fusion and detecting colocalized interacting proteins within single intact cells. a- and w-complementation can be exploited for a wide range of studies, including transgenic animals that express complementing lacZ mutants from two promoters of interest, which then could reveal cell lineages in which the products of both genes coincide spatially and temporally. In line with these results, Rossi et al. (48) applied b-galactosidase complementation to monitor protein–protein interactions in intact eukaryotic cells.
Given the wide range of applications for b-galactosidase during the last decades, one can anticipate that further development of the b-galactosidase fusion and complementation systems will contribute to our understanding of many features of cellular regulation and organization.
References
1.M. Cohn, J. Monod, H. R. Pollock, S. Spiegelman, and R. Stanier (1953) Nature 172, 1096–1097.
2.J. Monod, G. Cohen-Bazire, and M. Cohn (1951) Biochem. Biophys. Acta 7, 585–599.
3. J. Lederberg and E. L. Tatum (1946) Cold Spring Harbor Symp. Quant. Biol. 11, 113–114.
4.E. L. Wollman, F. Jacob, and W. Hayes (1956) Cold Spring Harbor Symp. Quant. Biol. 21, 141-162.
5.H. W. Rickenberg, G. N. Cohen, G. Buttin, and J. Monod (1956) Ann. Inst. Pasteur 91, 829–857.
857. 6I. Zabin and A. V. Fowler (1978) In The Operon (J. H. Miller and W. S. Reznikoff, eds.), Cold Spring Harbor Laboratory, Cold Spring Harbor, New York, pp. 89–121.
7. A. B. Pardee, F. Jacob, and J. Monod (1959) J. Mol. Biol. 1, 165–178.
8.F. Jacob and J. Monod (1961) J. Mol. Biol. 3, 318–356.
9.M. Cohn (1957) Bact. Rev. 21, 140–168.
10.K. Wallenfels and O. P. Malhotra (1961) Adv. Carbohydrate Chem. 16, 239–298.
11.A. Ullmann, F. Jacob, and J. Monod (1968) J. Mol. Biol. 32, 1–13.
12.A. Ullmann and J. Monod (1969) Biochem. Biophys. Res. Commun. 35, 35–42.
13.C. Burstein, M. Cohn, A. Kepes, and J. Monod (1965) Biochem. Biophys. Acta 95, 634–639.
14.M. Ring and R. E. Huber (1990) Arch. Biochem. Biophys. 283, 342–350.
15.C. G. Cupples, J. H. Miller, and R. E. Huber (1990) J. Biol. Chem. 265, 5512–5518.
16.R. H. Jacobson, X-J. Zhang, R. F. DuBose, and B. W. Matthews (1994) Nature 369, 761–766.
17.A. V. Fowler and I. Zabin (1977) Proc. Natl. Acad. Sci. USA 74, 1507–1510.
18.A. Kalnins, K. Otto, U. Rüther, and B. Müller-Hill (1983) EMBO J. 2, 593–597.
19.H. W. Stokes, P. W. Betts, and B. G. Hall (1985) Mol. Biol. Evol. 2, 469–477.
20.A. Ullmann and D. Perrin (1970) In The Lactose Operon (J. R. Beckwith and D. Zipser, eds.), Cold Spring Harbor Laboratory, Cold Spring Harbor, New York, pp. 143–172.
21.I. Zabin (1982) Mol. Cell. Biochem. 49, 87–96.
22.D. Perrin (1963) Cold Spring Harbor Symp. Quant. Biol. 28, 529–532.
23.F. H. C. Crick and L. E. Orgel (1964) J. Mol. Biol. 8, 161–165.
24.B. Rotman and F. Celada (1968) Proc. Natl. Acad. Sci. USA 60, 660–667.
25.E. Conway de Macario, J. Ellis, R. Guzman, and B. Rotman (1978) Proc. Natl. Acad. Sci. USA 75, 720-724.
26.F. Jacob, A. Ullmann, and J. Monod (1965) J. Mol. Biol. 31, 704–719.
27. A. Ullmann, D. Perrin, F. Jacob, and J. Monod (1965) J. Mol. Biol. 12, 918–923.
28.J. R. Beckwith (1964) J. Mol. Biol. 8, 427–430.
29.A. Cook and J. Lederberg (1962) Genetics 47, 1335–1353.
30.A. Ullmann, F. Jacob, and J. Monod (1968) J. Mol. Biol. 24, 339–343.
31.S. L. Morrison and D. Zipser (1970) J. Mol. Biol. 50, 359–371.
32. K. E. Langley, A. V. Fowler, and I. Zabin (1975) J. Biol. Chem. 250, 2587–2592.
33. K. E. Langley, M. R. Villarejo, A. V. Fowler, P. J. Zamenhof, and I. Zabin (1975) Proc. Natl. Acad. Sci. USA 72, 1254–1257.
34. R. S. Accolla and F. Celada (1976) FEBS Lett. 67, 299–302.
35. M. E. Goldberg and S. J. Edelstein (1969) J. Mol. Biol. 46, 431–440.
36.A. Ullmann and J. Monod (1970) in The Lactose Operon (J. R. Beckwith and D. Zipser, eds.), Cold Spring Harbor Laboratory, Cold Spring Harbor, New York, pp. 265–272.
37. F. Celada, A. Ullmann, and J. Monod (1974) Biochemistry 13, 5543–5547.
38. M. E. Goldberg (1969) J. Mol. Biol. 46, 441–446.
39. B. Müller-Hill and J. Kania (1974) Nature 249, 561–563.
40. T. J. Silhavy and J. Beckwith (1985) Microbiol. Rev. 49, 398–418.
41. J. R. Sanes, J. L. R. Rubenstein, and J-F. Nicolas (1986) EMBO J. 5, 3133–3142.
42. A. Ullmann (1984) Gene 29, 27–31.
43. U. Rüther, M. Koenen, K. Otto, and B. Müller-Hill (1981) Nucleic Acids Res. 9, 4087–4098.
44. J. Messing, B. Gronenborn, B. Müller-Hill, and P.H. Hofschneider (1977) Proc. Natl. Acad. Sci. USA 74, 3642–3646.
45. F. Sanger, S. Nicklen, and A. R. Coulson (1977) Proc. Natl. Acad. Sci. USA 74, 5463–5467.
46. J. Vieira and J. Messing (1982) Gene 19, 259–268.
47. W. A. Mohler and H. M. Blau (1996) Proc. Natl. Acad. Sci. USA 93, 12423–12427.
48. F. Rossi, C. A. Charlton, and H. M. Blau (1997) Proc. Natl. Acad. Sci. USA 94, 8405–8410.
الاكثر قراءة في مواضيع عامة في الاحياء الجزيئي
اخر الاخبار
اخبار العتبة العباسية المقدسة
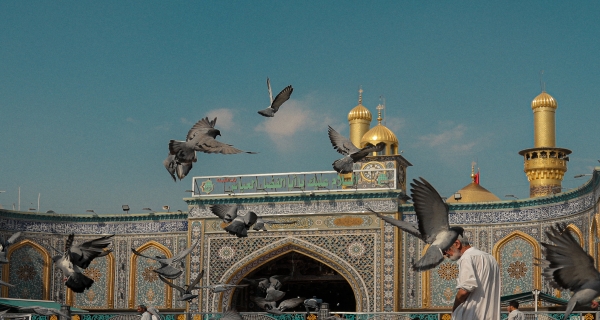
الآخبار الصحية
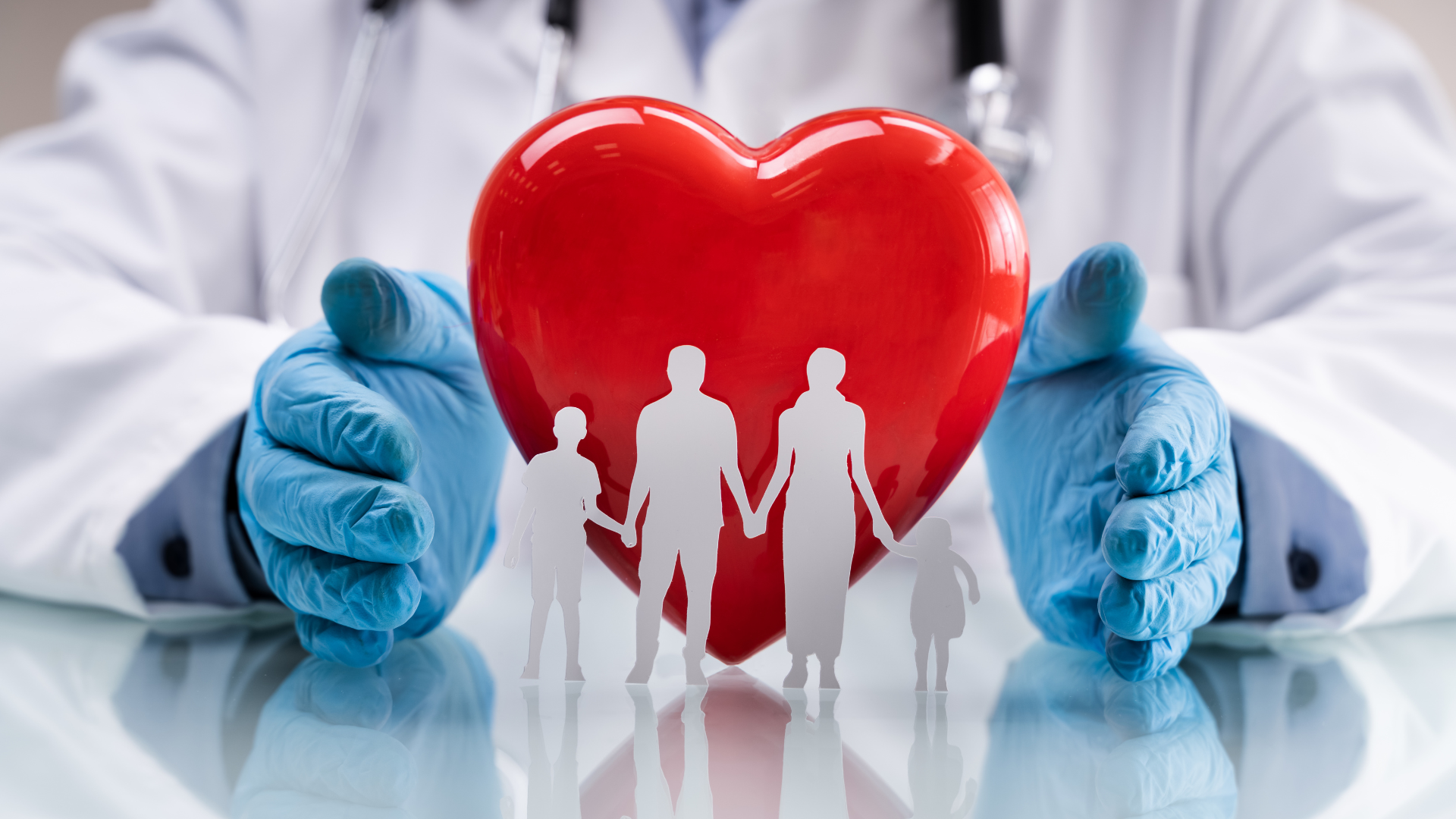